
Effects of an Herbal Medicinal Product Composed of Three Herbal Materials on Lipopolysaccharide-induced Depression-like Behaviors in Mice
Abstract
An herbal medicinal product consisting of three kinds of herbal materials, Prunella vulgaris L. (Lamiaceae), Clematis chinensis Osbeck (Ranunculaceae) and Trichosanthes kirilowii Max. (Cucurbitaceae) has been prescribed in the clinic for treating rheumatoid arthritis in Korea. In the present study, we investigated the antidepressive effect of this herbal complex extract (HCE) on lipopolysaccharide (LPS)-induced depression-like behavior. The effects of HCE on LPS-induced depressive-like behaviors were evaluated using a forced swimming test (FST) and splash test. In addition, we also evaluated locomotor activity and anxiety-like behaviors using the open field test and elevated plus-maze (EPM) test. Inflammatory cytokines were evaluated in the cortical regions. HCE attenuated anxiety-like behavior in the EPM test and depressive- and anhedonia-like behaviors induced by LPS in the FST and splash test. In addition, LPS-induced increases in the phosphorylation levels of protein kinase B (Akt) and glycogen synthase kinase 3 beta (GSK-3β) and expression levels of proinflammatory factors in the cortex were normalized by HCE. Moreover, decreases in the level of BDNF in the cortex were attenuated by HCE. These results suggest that HCE attenuates inflammation-induced depression-like behaviors through its normalization of Akt-GSK-3β signaling and proinflammatory factors and its upregulation of BDNF in the cortex and that HCE has therapeutic potential for depressive disorders in inflammatory states.
Keywords:
Herbal medicinal product, Depressive disorder, Lipopolysaccharide, Proinflammatory factor, BDNF, Akt-GSK-3β signalingIntroduction
Depressive disorders impact approximately 4.4% of the global population, and disability from this disorder accounted for 7.5% of all causes of global disability in 2015.1 The pathological mechanism of depression or its biomarker is still unclear, and the current diagnosis of depressive patients has focused on their mood and cognitive symptoms according to the Hamilton depression rating scale (HAM-D) scores and Manual of Diagnosis and Statistics of Mental Disorders (DSM-V) criteria.2–4 Current therapeutics for depression are categorized by selective serotonin reuptake inhibitors (SSRIs), serotonin and norepinephrine reuptake inhibitors (SNRIs), monoamine oxidase inhibitors (MAOIs) and other mechanisms. Such antidepressants effectively reverse affective mood disorders or cognitive dysfunctions.5,6 However, recent studies suggest that depressive patients have poor drug adherence due to its adverse effects, such as drowsiness, nausea or vomiting and sexual problems.7 Therefore, new anti-depressive drugs with fewer adverse effects are urgently needed.
It has been acknowledged that the number of inflammatory markers increases in patients with depression.8–11 Furthermore, several clinical studies have shown that anti-inflammatory drugs, such as nonsteroidal anti-inflammatory drugs (NSAIDs) or cytokine inhibitors, have a therapeutic effect on depressive-like disorders.12–14 Several mouse models with depressive disorders also showed overexpression of proinflammatory cytokines, including interleukin (IL)-1β and tumor necrosis factor (TNF)-α in blood and/or brain tissues of the prefrontal cortex (PFC) and hippocampal regions, indicating that inflammatory cytokines play important roles in the causes or maintenance of depression.15–17 Similarly, direct inhibition of IL-1β expression also had a therapeutic effect on anxiety- and depression-like behaviors induced by lipopolysaccharide (LPS) in mice.18 These results suggest that a new drug with anti-inflammatory activity and fewer adverse effects might be a promising agent if it exhibits anti-depressive activities. We aim to find new drugs from herbal materials for anti-depressants because of their fewer adverse effects and low costs associated with normal pharmacotherapy.19–23 In addition, we are attempting to develop new drugs by repurposing herbal prescriptions that exhibit anti-inflammatory activity.
JOINS® (SKI306X, SK Chemicals, Seongnam, Korea), an herbal medicinal product, has been prescribed in the clinic for treating rheumatoid arthritis in Korea for a few years and consists of three kinds of herbal materials, Prunella vulgaris L. (Lamiaceae), Clematis chinensis Osbeck (Ranunculaceae), and Trichosanthes kirilowii Max. (Cucurbitaceae). It is well known that JOINS® exerts good antipyretic, analgesic, and anti-inflammatory effects.24,25 Interestingly, it has been reported that rheumatoid arthritis is associated with incidence of depressive disorders.26,27 Therefore, we assumed that JOINS®, as an anti-inflammatory agent, would be a beneficial agent for treating depressive-like disorders if its anti-inflammatory activity is also exhibited in the brain.
In this study, we aimed to probe whether the herbal complex extract (HCE), or its main active compound, rosmarinic acid (RA), could ameliorate LPS-induced depression-like behaviors in a mouse model. We employed several behavioral tests, including the forced swimming test, and explored the possible mechanism of HCE.
Experimental
Animals – Five-week-old male ICR mice weighing 25–30 g were purchased from Orient Co. Ltd., a branch of Charles River Laboratories (Seongnam-si, Korea). All mice were maintained with free access to water and food at a constant temperature (23±1℃) and humidity (60±10%) under a 12 h light and dark cycle (light on at 7:30 a.m.) at the University Animal Care Unit for one week before all experiments. All experimental protocols using animals were approved by the IACUC of Kyung Hee University (KHSASP-20-340).
Materials – RA, LPS and desipramine were purchased from Sigma-Aldrich Chemical Co. (St. Louis, MO). Anti-IL-1β, anti-IL-6, anti-TNF-α, anti-cyclooxygenase-2 (COX-2), anti-nuclear factor kappa-light-chain-enhancer of activated B cells (NF-κB), anti-protein kinase B (Akt), anti-glycogen synthase kinase-3β (GSK-3β), anti-β-actin, and anti-tropomyosin receptor kinase B (TrkB) antibodies were purchased from Santa Cruz Biotechnology, Inc. (Santa Cruz, CA). Anti-phosphorylated-GSK-3β antibodies were purchased from Cell Signaling Technology (Danvers, MA). Anti-brain-derived neurotrophic factor (BDNF) antibody was purchased from Abcam (Cambridge, UK). Anti-phosphorylated-TrkB antibody was purchased from Millipore Co. (Burlington, MA). Anti-phosphorylated-Akt antibody and all secondary antibodies were purchased from GeneTex, Inc. (Irvine, CA). The HCE prepared in our previous work was used in the present study.28 In brief, each herbal material (C. chinensis, 30 g; P. vulgaris, 30 g; T. kirilowii, 60 g) was purchased from Edam Pharm. Co. (Seoul, Korea) and extracted twice with 30% ethanol (1.2 L) under reflux (60℃) for 4 h. After filtration, the extract was concentrated and partitioned with n-butanol three times. The n-butanol fractions obtained were concentrated and freeze-dried into a powder (yield, 2.39±0.05%). The quality consistency of HCE was maintained by measuring the concentration of RA (average concentration was 14.35±0.04 mg/g) through HPLC analysis, as previously described.28
Drug administration and behavioral procedures – HCE was dissolved in 10% Tween 80 solution. RA, desipramine, and LPS were dissolved in 0.9% saline solution. The day before behavior tests, mice were injected with LPS (1.0 mg/kg, i. p.) for establishing an LPS-induced depressive-like animal model (Fig. 1). The next day, mice were administered HCE (30, 100, 300 mg/kg, p.o.), RA (1, 3, 10 mg/kg, p.o.) or vehicle solution 1 h before each behavior test and introduced into each designated behavioral test (Fig. 1). Desipramine (15 mg/kg, i. p.) as a positive control was injected 30 min before each test. All behavior tests were conducted during 10:00–17:00 under dim light and recorded by EthoVision system (Noldus, Wageningen, the Netherlands). All data were analyzed by a researcher who was well-trained and blind to each treatment.
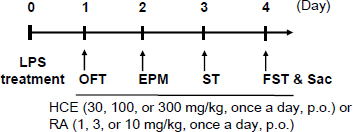
The experimental schedule of lipopolysaccharide (LPS) treatment and behavior tests. LPS (1.0 mg/kg, i.p.) was injected 1 day before the open field test (OFT). Each behavior experiment was conducted for 4 days from Day 1 after LPS administration (Day 0). After the completion of behavior tests, the animals were euthanized for mechanistic studies. OFT, open field test; EPM, elevated plus-maze test; ST, splash test; FST, forced swimming test.
Open field test (OFT) – The OFT for measuring spontaneous locomotor behavior was conducted as described previously.29 The OFT was performed in clean black Plexiglas boxes (W×D×H:41.5×41.5×41.5 cm) equipped with the video-based EthoVision System (Noldus, Wageningen, the Netherlands). Mice were initially introduced into the center of the box and allowed to freely explore the test box for 30 min (Day 1). Total distance moved and crossing of center zone (W×D:13.8×13.8 cm) were calculated for evaluating the effects of HCE and RA on spontaneous locomotor activity. The test box was wiped with 70% ethanol-soaked tissue between each trial.
Elevated plus-maze (EPM) test – The EPM was a cross-shaped apparatus 40 cm above the ground, which was used to measure anxiety-like behavior in rodents.30 The apparatus divided into four-elevated arms with quadrants of equal lengths (W×D:7×30 cm) which were radiated from a central platform (W×D:7×7 cm). The four-elevated arms consisted of two open arms and two closed arms (D×H:30×30 cm). During testing (Day 2), each mouse was placed on the central area with head-facing open arms. Free exploration behavior of each mouse was recorded for 5 min and the time spent in each arm during 5 min was analyzed by the video-based EthoVision System (Noldus, Wageningen, the Netherlands). The apparatus was cleaned prior to each trial using 70% ethanol solution.
Splash test – Splash test was used to detect anhedonia-like behaviors in mice, as described elsewhere.31,32 Mice were placed into the test cage (W×D×H:15.2×26.7×12.1 cm) and allowed to freely habituate the test cage for 1 min. After habituation, the prepared 10% sucrose solution was evenly sprayed on the dorsal of the mouse (0.5 mL), and the grooming time was manually measured during 5 min (Day 3). At the end of each trial, mice were wiped with clean water, and the test box was wiped using 70% ethanol spray.
Forced swimming test (FST) – The FST was conducted, as described previously 30. Mice were put into a glass cylinder sink (H×W:25×14 cm), filled with 20 cm fresh water (24±2℃), and allowed to swim for 6 min (Day 4). The swimming behaviors of each mouse were recorded by video camera. The feces of mice floating above the sink were fished out between each trial. The time of immobility, defined as few movements as necessary to keep its head above water, was analyzed during the last 4 min during 6 min using a video-based EthoVision System (Noldus, Wageningen, the Netherlands).
Western blot – Mice were sacrificed immediately after the forced swimming test (Day 4) and their brains were removed. The whole cortex and hippocampus were isolated from the removed brain and homogenized in Tris-HCl buffer (20 mM, pH 7.4). Homogenates were centrifuged at 14,000 rpm for 20 min at 4℃ and the supernatant was harvested. The sample which was quantified containing 15 μg of protein were separated through 6–12% sodium dodecyl sulfate-polyacrylamide gel electrophoresis (SDS-PAGE) gels under reducing situation and subsequently transferred to polyvinylidene fluoride (PVDF) membranes at 300 mA for 2 h. Then, the PVDF membranes were incubated for 2 h with blocking solution (5% skim milk) at room temperature. Subsequently, the samples were incubated overnight with diluted anti-IL-1β, IL-6, TNF-α, COX-2, NF-κB, BDNF, pTrkB, or TrkB (1:1000 dilution in 3% skim milk) and pAkt, Akt, pGSK-3β, GSK-3β, or β-actin (1:3000 dilution) antibodies at 4 ℃ and washed six times with Tween 20/Tris-buffered saline (TTBS). Thereafter, the membranes were incubated with suitable secondary antibodies (1:5000 dilution) for 2 h at room temperature and washed with TTBS for 60 min. The membrane was developed with enhanced chemiluminescence and the immunoblot was analyzed using the Multigauge bio-imaging program on the LAS-4000 mini system (Fujifilm Life Science USA, Stamford, CT) with ImageJ software (NIH, Bethesda, MD).
Statistical analysis – Data were expressed as the means±standard errors of the means (S.E.M.). The data from splash test, EPM test, OFT, FST, and Western blot assay were analyzed by a one-way analysis of variance (ANOVA) followed by Newman-Keuls post hoc test. All behavioral data were analyzed by well-trained researchers who were blind to any treatment. The criterion for significance was set at p<0.05.
Results and discussion
We evaluated whether HCE or RA could alleviate LPS-induced depressive-like behavior through FST. One-way ANOVA revealed that there were significant group differences on the immobility time (HCE, F5,54=9.610, p<0.05; RA, F5,55=6.957, p<0.05) (Fig. 2A and B). A single injection of LPS both in the HCE or RA treatment experiments extended immobility time with significant difference compared to control group (p<0.0001), indicating that the depressive-like mice model was constructed successfully after LPS injection. HCE (100 mg/kg) or RA (3 mg/kg)-treated mice struggled and decreased immobility time in FST (p<0.0001), as observed in desipramine-treated group.
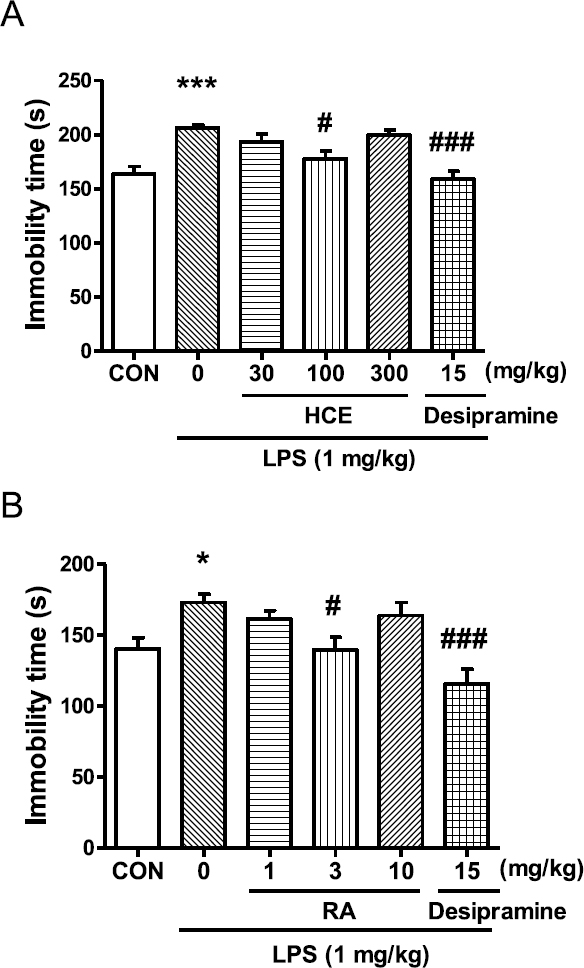
Effects of herbal complex extract (HCE) or rosmarinic acid (RA) on the LPS-induced depressive-like behaviors in the forced swimming test. HCE (30, 100, 300 mg/kg, p.o.), RA (1, 3, 10 mg/kg, p.o.), or vehicle (10% Tween 80 solution) was administered to mice. Desipramine (15 mg/kg, i.p.) was used as a positive control. Depressive-like disorder was induced by LPS (1 mg/kg). After habituation, HCE, RA, or vehicle was administered one hour before the test, and desipramine was administered 30 min before the test. The data represent the means±SEM (n=8-10 per group) of the immobility time. ***p<0.0001, vs. the control group treated with vehicle; #p<0.05, ###p<0.0001, vs. the LPS-treated group; CON, control.
To determine if HCE or RA reverses anhedonia-like behavior induced by LPS in mice, the splash test was employed and there were significant group differences on the grooming time (F5,53=8.467, p<0.05) (Fig. 3A). LPS treatment significantly decreased grooming time compared to normal group (p<0.05), showing that anhedonia-like behaviors could be induced by LPS.33 The administration of HCE (100 mg/kg) significantly increased grooming time compared to the LPS-treated group (p<0.05). In the case of RA treatment, one-way ANOVA showed that there were significant group differences in grooming time (F5,53=9.457, p<0.05) (Fig. 3B). Decreased grooming time by LPS treatment was increased by the administration of RA (3 mg/kg) (p<0.05). Nonetheless, desipramine could not reverse grooming time decreased by LPS treatment.
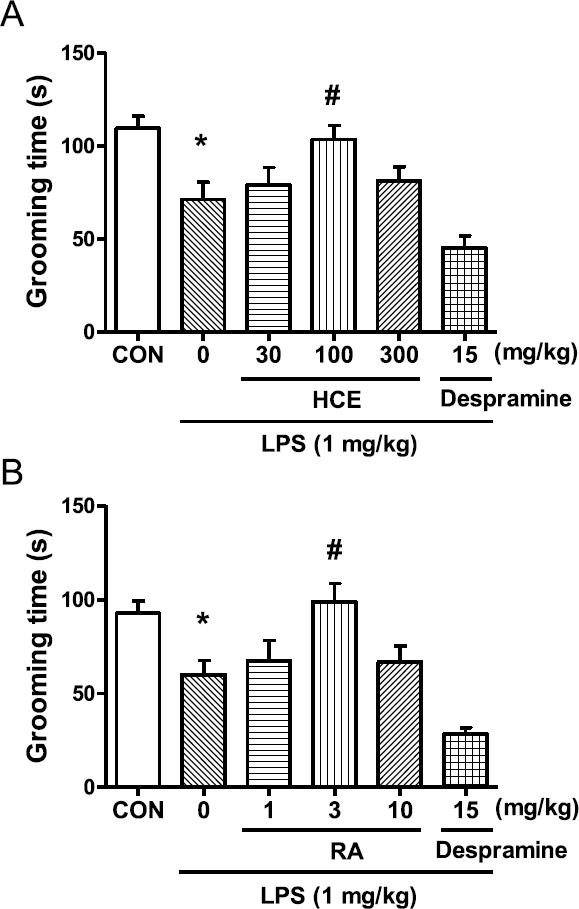
Effects of herbal complex extract (HCE) or rosmarinic acid (RA) on the LPS-induced anhedonia-like behaviors in the splash test. HCE (30, 100, 300 mg/kg, p.o.), RA (1, 3, 10 mg/kg, p.o.), or vehicle (10% Tween 80 solution) was administered to mice. Desipramine (15 mg/kg, i.p.) was used as a positive control. Anhedonia-like activity was induced by LPS (1 mg/kg). After habituation, HCE, RA or vehicle was administered one hour before the test, and desipramine was administered 30 min before the test. The data represent the means±SEM (n=9-10 per group) of grooming time. *p<0.05 vs. the control group treated with vehicle; #p<0.05, vs. the LPS-treated group; CON, control.
To examine the effects of HCE or RA on the anxiety-like behavior induced by LPS, mice were administered HCE or RA 1 h before the EPM test. One-way ANOVA presented a significant difference between groups on the percentage of time spent in the open arms (F5,53=2.813, p<0.05) (Fig. 4A). The time spent in open arms (%) was significantly decreased by LPS injection compared to normal group (p<0.05), suggesting that LPS injection induced an anxiety-like behavior.33 Furthermore, with the treatment with HCE (30, 100 or 300 mg/kg, p.o.) or desipramine (15 mg/kg, i.p.), the percentage of time spent in open arms was significantly increased compared to LPS-treated group, as did desipramine. In the case of RA, one-way ANOVA presented an obvious difference between groups on the percentage of time spent in the open arms (F5,54=2.992, p<0.05) (Fig. 4B). With the treatment with RA (3 and 10 mg/kg) and desipramine, the percentage of time spent in the open arms significantly increased compared to LPS-injected group, respectively.
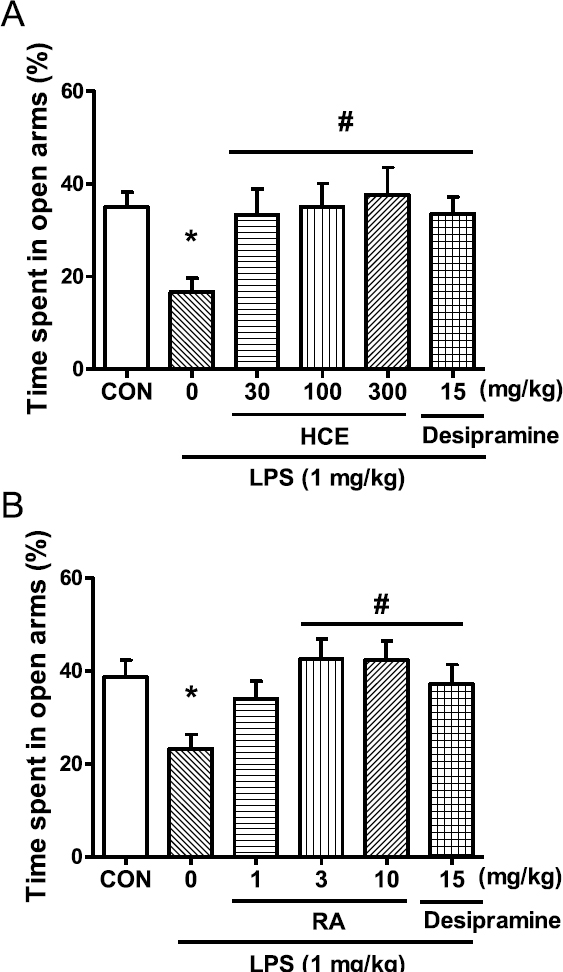
Effects of herbal complex extract (HCE) or rosmarinic acid (RA) on the LPS-induced anxiety-like behaviors in the elevated plus-maze test. HCE (30, 100, 300 mg/kg, p.o.), RA (1, 3, 10 mg/kg, p.o.), or the vehicle (10% Tween 80 solution) was administered to mice. Desipramine (15 mg/kg, i.p.) was used as a positive control. Anxiety-like activity was induced by LPS (1 mg/kg). After habituation, HCE, RA or vehicle was administered one hour before the test, and desipramine was administered 30 min before the test. The data represent the means±SEM (n=9-10 per group) of the duration in the open arms. ***p<0.0001, **p<0.001, *p<0.05 vs. the control group treated with vehicle; #p<0.05, vs. the LPS-treated group; CON, control
To investigate the effect of HCE or RA on hypo-locomotor behavior induced by LPS, an OFT was conducted. One-way ANOVA revealed that there was a significant group difference on the moved distance and the crossing number of center zone (distance moved, F5,48=18.08, p<0.05; crossing number of center zone, F5,52=9.574, p<0.05) (Fig. 5A and B). In contrast to control group, the LPS-treated group showed significantly fewer number of center zone crossing (p<0.05) and decreased total distance moved in 30 min (p<0.05). HCE slightly reversed the decrease of total moving distance induced by LPS at 100 and 300 mg/kg but not significantly, as desipramine did not. In the case of RA, one-way ANOVA revealed that there was a significant group effect between the groups (distance moved, F5,54=13.77, p<0.05; crossing number of center zone, F5,53=3.204, p<0.05) (Fig. 5D and E). The LPS-treated group showed significantly fewer times of center zone crossing (p<0.05) and decreased total distance moved in 30 min (p<0.05). As observed in HCE administration group, RA slightly reversed the decrease of total moving distance induced by LPS at 3 and 10 mg/kg but not significantly.
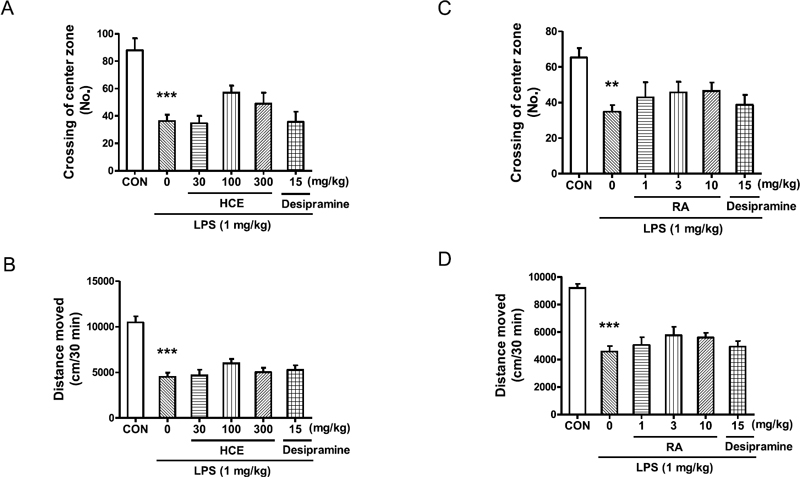
Effects of herbal complex extract (HCE) or rosmarinic acid (RA) on the LPS-induced hypo-locomotor behaviors in the open field test. HCE (30, 100, 300 mg/kg, p.o.), RA (1, 3, 10 mg/kg, p.o.), or vehicle (10% Tween 80 solution) was administered to mice Hypo-locomotor behavior was induced by LPS (1 mg/kg). After habituation, HCE, RA or vehicle was administered one hour before the test, and desipramine (15 mg/kg, i.p.) used as positive control was administered 30 min before the test. The crossing of center zone (A, HCE; C, RA), and total distance moved (cm) in 30 min (B, HCE; D, RA are exhibited. The data represent the means±SEM (n=8-10 per group) ***p<0.0001, vs. the control group treated with vehicle; CON, control.
We examined the phosphorylation levels of Akt, GSK-3β or TrkB, or the level of BDNF expression after administration of HCE in the cortical region. The expression levels of each signaling molecule were analyzed in the cortex (pAkt, F5,30=5.200, p=0.015; pGSK-3β, F5,30=10.26, p<0.0001; pTrkB, F5,30=2.737, p=0.0375; BDNF, F5,30=2.912, p=0.0341). As shown in Fig. 6, LPS treatment markedly increased the levels of pAkt (p<0.01) and pGSK-3β (p<0.001) and decreased BDNF expression levels and its receptor phosphorylation (p<0.05). The increased levels of pAkt and pGSK-3β, and decreased BDNF expression in the cortex were significantly reversed by the HCE administration (100 or 300 mg/kg). However, pTrkB showed only a tendency of increase in HCE administration, but not significantly.
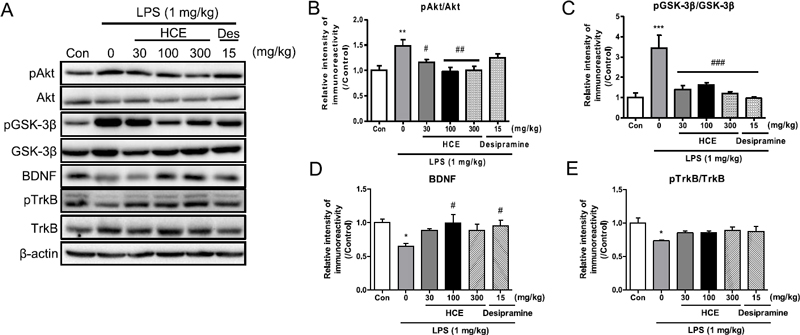
Effects of herbal complex extract (HCE) on the LPS-induced phosphorylation levels of Akt, GSK-3β, and TrkB and the expression level of BDNF in the cortex. HCE (30, 100, 300 mg/kg, p.o.) or vehicle (10% Tween 80 solution) was administered one hour before the test. Desipramine (Des) used as positive control was administered 30 min before sacrificing the mice. The data represent the means±SEM (n=6 per group). Immunoreactivity was normalized to control (taken as 1.0). *p<0.05, vs. the control group; **p<0.01, vs. the control group; ***p<0.001, vs. the control group; #p<0.05, vs. the LPS-treated group; ##p<0.01, vs. the LPS-treated group; ###p<0.001, vs. the LPS-treated group; CON, control.
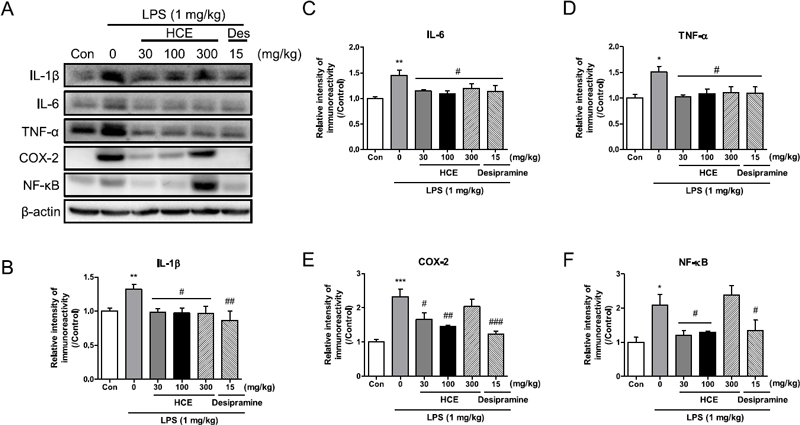
Effects of herbal complex extract (HCE) on the LPS-induced upregulation levels of IL-1β, IL-6, TNF-α, COX-2 and NF-κB in the cortex. HCE (30, 100, 300 mg/kg, p.o.) or vehicle (10% Tween 80 solution) was administered to mice with same drug dosage. HCE or vehicle was administered one hour before the test, and desipramine (Des) employed as positive control was administered 30 min before sacrificing the mice. The data represent the means±SEM (n=5-6 per group). Immunoreactivity was normalized to control (taken as 1.0). *p<0.05, vs. the control group; **p<0.01, vs. the control group; ***p<0.001, vs. the control group; #p<0.05, vs. the LPS-treated group; ##p<0.01, vs. the LPS-treated group; ###p<0.001, vs. the LPS-treated group; CON, control.
To observe the change of inflammation-associated factors induced by LPS, we investigated the expression levels of inflammatory cytokines after the administration of HCE in the cortex (IL-1β, F5,30=3.327, p=0.0165; IL-6, F5,30=3.865, p=0.008; TNF-α, F5,29=3.865, p=0.0104; COX-2, F5,29=10.10, p<0.0001; NF-κB, F5,30=5.895, p=0.0007). LPS treatment could activate inflammatory reactions through the activation of toll-like receptor 4 (TLR4) which induces the activation of NF-κB, and activated NF-κB increased pro-inflammatory factors including IL-1β, IL-6, or TNF-α.34 As expected, levels of IL-6, TNF-α, COX-2, and NF-κB were significantly increased after administration of LPS (p<0.05, p<0.01 or p<0.001). The above inflammation-associated factors were normalized by HCE (100 mg/kg) administration, as observed in desipramine treatment. However, levels of COX-2 and NF-κB were unchanged by the high dose of HCE (300 mg/kg).
In the present study, we attempted to explore whether HCE as an anti-inflammatory agent has anti-depressive-like activity under a drug repositioning strategy. We observed that the administration of HCE could ameliorate LPS-induced depression- and anxiety-like symptoms. In addition, HCE reversed the increased levels of IL-1β, IL-6, TNF-α, COX-2, and NF-κB expression induced by LPS injection and normalized the levels of pAkt, pGSK-3β, and BDNF expression.
Several lines of evidence suggest that the levels of inflammatory factors in patients with depression have a high correlation with depression.8,35–39. LPS promotes secretion of proinflammatory cytokines, nitric oxide, and eicosanoids and is typically used for establishing a depressive-like animal model.40,41 In our present study, increased immobility time and decreased time spent in the open arms and grooming time were observed after LPS administration, indicating that the LPS-induced depressive mouse model is useful for determining depressive-like behaviors. Previously, we reported that HCE ameliorates schizophrenia-like symptoms in mice,28 suggesting that HCE or its active constituents could penetrate the brain-blood barrier and exert its pharmacological activities. Meanwhile, recent studies suggest that immune activation characterized by the upregulation of major proinflammatory cytokines plays a role in the pathogenesis of schizophrenia.36,42,43 Therefore, this finding reveals that both schizophrenia and depression are associated with increased inflammatory cytokines. This led us to reconsider whether HCE with anti-inflammatory effects in the brain has a therapeutic effect on depressive-like symptoms, and our research may extend the clinical applicability of JOINS®. Additionally, long-term use of HCE containing RA has already been reported in clinical trial.44 In the present study, we observed that HCE ameliorated the deficits in the EPM, FST, and splash test, showing that HCE could be beneficial in treating depression-like symptoms including anxiety and anhedonia symptoms.
Next, we investigated which compounds might be responsible for the antidepressive-like behaviors of HCE. Although several compounds are contained in the HCE, we employed RA to maintain the quality consistency of HCE because of its high amount in HCE. RA has been known to have anti-inflammatory or antioxidant actions.45,46 Recently, it has been reported that LPS induced depressive-like symptoms were attenuated by treatment with RA in mice.47 Similar behavioral outcomes were also observed in our study, although the dose ranges were different. RA administration (3 mg/kg, p.o.) changed depressive-like, anxiety-like and anhedonia-like behaviors induced by LPS (1 mg/kg) in mice, and RA treatment (10 mg/kg) significantly increased the duration of open arms in the EPM test. Therefore, our present study demonstrated that HCE, or its main constituent RA, would be effective for treating the depressive-like, anxiety-like, and anhedonia-like symptoms observed in patients with depression. However, considering the active dosage of RA and HCE, RA might not solely be responsible for the anti-depression- or anxiety-like activity of HCE. Oleanolic, ursolic acid, and its glycoside are also contained in HCE, and such compounds are also reported to have antidepressive activity.48–50 Interestingly, HCE administration showed an inverted U-shaped dose-response pattern in behavioral studies. Similar results were also observed for the expression levels of COX-2 and NF-κB (vide supra). Although we cannot explain these results, it can be speculated as follows: A high dose of HCE (300 mg/kg) or RA (10 mg/kg) is likely to decrease neurotransmitters that are associated with anti-depressive activities through their autoreceptors.51 Further research is needed to clarify this issue.
It has been reported that the Akt-GSK-3β pathway is activated in a depressive-like behavior mouse model52 and that activating this pathway restrains the expression level of BDNF.53 Additionally, the peripheral levels of BDNF were decreased in patients with depression.54 Akt is known to be activated by phosphoinositide 3-kinase (PI3K) through phosphorylation and restrains GSK-3β, which regulates cell survival.55 In addition, several studies have showed that the expression of BDNF and phosphorylation levels of TrkB are decreased by activation of the Akt-GSK-3β pathway,56 which causes a lack of neuroplasticity or cell death.57 Therefore, we investigated the changes of Akt-GSK-3β signaling and the expression level of BDNF in the LPS-induced depression mouse model. In the LPS-injected group, the phosphorylation level of the Akt-GSK-3β pathway was increased, and the expression level of BDNF was decreased. In contrast, the administration of HCE (100 mg/kg) significantly normalized the Akt-GSK-3β signaling pathway and increased the expression level of BDNF, although the TrkB activity decreased by LPS administration was not recovered by HCE administration. Taken together, the present studies suggest that HCE could ameliorate Akt-GSK-3β and BDNF signaling, which was impaired by LPS administration.
A growing number of studies have investigated to support the inflammatory hypothesis on the pathogenesis and treatment of depressive disorder. For example, chronic stress upregulates proinflammatory cytokines by activating IL-1β and IL-18 and autophagy system in the prefrontal cortical region.58 In addition, LPS activates the Akt-GSK-3β signaling pathway, which could promote inflammation.59 Meanwhile, growing evidence has demonstrated that inhibition of the IL-1β receptor can reduce the occurrence of depressive-like behaviors in rodents, indicating that regulation of inflammatory cytokines would be a novel strategy for developing anti-depressant agents.60 In our present study, we also examined the effects of HCE on the expression levels of IL-1β, IL-6, TNF-α, NF-κB, and COX-2 in the cortex of mice. HCE ameliorated LPS-induced proinflammatory cytokine expression, including IL-1β, IL-6, TNF-α and COX-2. In addition, in the HCE-treated group, the expression level of NF-κB was significantly decreased compared with that in the LPS-treated group. These results suggest that HCE is able to inhibit the inflammatory reaction induced by LPS, and, thereafter, decrease pro-inflammatory factors. We investigated whether LPS treatment-induced microglial or astrocytic activation in the cortical or hippocampal regions to identify the origin of proinflammatory factors. However, we did not observe any changes in allograft inflammatory factor 1 (Iba-1), a marker of microglia, or glial fibrillary acidic protein (GFAP), a marker of astrocytes, immunoreactivities after the administration of LPS. It was reported that activated glial cells secrete cytokines after the initial phase of inflammation.61,62 Additionally, it was reported that the levels of inflammatory cytokines, such as IL-6 or IL-1β, were higher at 5 or 6 days after exposure to proinflammatory agents compared to the day after the exposure to pro-inflammatory agents.63 These results suggested that HCE could inhibit the late stages of LPS-induced inflammatory responses.
In summary, HCE or its active compound, RA, administration attenuated LPS-induced depressive-, anxiety-, and anhedonia-like symptoms. These effects of HCE would be associated with its anti-inflammatory activity and its increase activity on BDNF expression level through Akt-GSK-3β pathway in the cortex. Collectively, our findings on the anti-depressant activity of HCE suggest that HCE would be useful for treating depressive patients with inflammation-associated symptoms.
Acknowledgments
This research was supported by a grant of the Korea Health Technology R&D Project through the Korea Health Industry Development (KHIDI), funded by the Ministry of Health &welfare, Republic of Korea (RS-2022-KH127677), and this research was supported by Korea Drug Development Fund funded by Ministry of Science and ICT, Ministry of Trade, Industry, and Energy, and Ministry of Health and Welfare (RS-2021-DD120983, Republic of Korea).
Conflicts of Interest
The authors declare that they have no conflicts of interest.
References
-
Otte, C.; Gold, S. M.; Penninx, B. W.; Pariante, C. M.; Etkin, A.; Fava, M.; Mohr, D. C.; Schatzberg, A. F. Nat. Rev. Dis. Primers 2016, 2, 16065.
[https://doi.org/10.1038/nrdp.2016.65]
-
Andrews, G.; Slade, T.; Sunderland, M.; Anderson, T. Am. J. Psychiatry. 2007, 164, 1784–1785.
[https://doi.org/10.1176/appi.ajp.2007.07060928]
-
Insel, T. R.; Cuthbert, B. N. Science 2015, 348, 499–500.
[https://doi.org/10.1126/science.aab2358]
-
Zimmerman, M.; Galione, J. N.; Chelminski, I.; Young, D.; Dalrymple, K.; Witt, C. F. Depress. Anxiety 2010, 27, 977–981.
[https://doi.org/10.1002/da.20710]
-
Baune, B. T.; Renger, L. Psychiatry Res. 2014, 219, 25–50.
[https://doi.org/10.1016/j.psychres.2014.05.013]
-
Nelson, J. C.; Delucchi, K. L.; Schneider, L. S. Am. J. Psychiatry. 2013, 170, 651–659.
[https://doi.org/10.1176/appi.ajp.2012.12070927]
-
Read, J.; Williams, J. Curr. Drug Saf. 2018, 13, 176–186.
[https://doi.org/10.2174/1574886313666180605095130]
-
Dowlati, Y.; Herrmann, N.; Swardfager, W.; Liu, H.; Sham, L.; Reim, E. K.; Lanctôt, K. L. Biol. Psychiatry 2010, 67, 446–457.
[https://doi.org/10.1016/j.biopsych.2009.09.033]
-
Hou, R.; Ye, G.; Liu, Y.; Chen, X.; Pan, M.; Zhu, F.; Fu, J.; Fu, T.; Liu, Q.; Gao, Z.; Baldwin, D. S.; Tang, Z. Brain Behav Immun. 2019, 81, 105–110.
[https://doi.org/10.1016/j.bbi.2019.06.001]
-
Osimo, E. F.; Pillinger, T.; Rodriguez, I. M.; Khandaker, G. M.; Pariante, C. M.; Howes, O. D. Brain Behav. Immun. 2020, 87, 901–909.
[https://doi.org/10.1016/j.bbi.2020.02.010]
- Zhou, L.; Ma, X.; Wang, W. Neuropsychiatr. Dis. Treat. 2020, 16, 81–86.
-
Iyengar, R. L.; Gandhi, S.; Aneja, A.; Thorpe, K.; Razzouk, L.; Greenberg, J.; Mosovich, S.; Farkouh, M. E. Am. J. Med. 2013, 126, 1017. e1011–1018.
[https://doi.org/10.1016/j.amjmed.2013.02.037]
-
Köhler, O.; Benros, M. E.; Nordentoft, M.; Farkouh, M. E.; Iyengar, R. L.; Mors, O.; Krogh, J. JAMA Psychiatry. 2014, 71, 1381–1391.
[https://doi.org/10.1001/jamapsychiatry.2014.1611]
- Na, K.-S.; Lee, K. J.; Lee, J. S.; Cho, Y. S.; Jung, H.-Y. Prog. Neuropsychopharmacol. Biol. Psychiatry 2014, 48, 79–85.
-
Bollinger, J. L.; Horchar, M. J.; Wohleb, E. S. Neuropsychopharmacology 2020, 45, 1766–1776.
[https://doi.org/10.1038/s41386-020-0720-1]
-
Farooq, R. K.; Asghar, K.; Kanwal, S.; Zulqernain, A. Biomed. Rep. 2017, 6, 15–20.
[https://doi.org/10.3892/br.2016.807]
-
Wang, J.; Hodes, G. E.; Zhang, H.; Zhang, S.; Zhao, W.; Golden, S. A.; Bi, W.; Menard, C.; Kana, V.; Leboeuf, M.; Xie, M.; Bregman, D.; Pfau, M. L.; Flanigan, M. E.; Esteban-Fernández, A.; Yemul, S.; Sharma, A.; Ho, L.; Dixon, R.; Merad, M.; Han, M.-H.; Russo, S. J.; Pasinetti, G. M. Nat. Commun. 2018, 9, 477.
[https://doi.org/10.1038/s41467-017-02794-5]
-
Li, M.; Li, C.; Yu, H.; Cai, X.; Shen, X.; Sun, X.; Wang, J.; Zhang, Y.; Wang, C. J. Neuroinflammation. 2017, 14, 190.
[https://doi.org/10.1186/s12974-017-0964-9]
-
Kessler, D.; Bennewith, O.; Lewis, G.; Sharp, D. BMJ 2002, 325, 1016–1017.
[https://doi.org/10.1136/bmj.325.7371.1016]
-
Knüppel, L.; Linde, K. J. Clin. Psychiatry 2004, 65, 1470–1479.
[https://doi.org/10.4088/JCP.v65n1105]
-
Sarris, J. Phytother. Res. 2018, 32, 1147–1162.
[https://doi.org/10.1002/ptr.6055]
-
Schulz, V. Phytomedicine 2006, 13, 199–204.
[https://doi.org/10.1016/j.phymed.2005.07.005]
-
Yeung, W.-F.; Chung, K.-F.; Ng, K.-Y.; Yu, Y.-M.; Ziea, E. T.-C.; Ng, B. F.-L. J. Psychiatr. Res. 2014, 57, 165–175.
[https://doi.org/10.1016/j.jpsychires.2014.05.016]
-
Hartog, A.; Hougee, S.; Faber, J.; Sanders, A.; Zuurman, C.; Smit, H. F.; van der Kraan, P. M.; Hoijer, M. A.; Garssen, J. Phytomedicine 2008, 15, 313–320.
[https://doi.org/10.1016/j.phymed.2007.09.005]
-
Kim, H.-R.; Kim, K.-W.; Kim, B.-M.; Won, J.-Y.; Min, H.-K.; Lee, K.-A.; Kim, T.-Y.; Lee, S.-H. J. Clin. Med. 2019, 8, 1012.
[https://doi.org/10.3390/jcm8071012]
-
Marrie, R. A.; Hitchon, C. A.; Walld, R.; Patten, S. B.; Bolton, J. M.; Sareen, J.; Walker, J. R.; Singer, A.; Lix, L. M.; El-Gabalawy, R.; Katz, A.; Fisk, J. D.; Bernstein, C. N. Arthritis Care Res. (Hoboken). 2018, 70, 970–978.
[https://doi.org/10.1002/acr.23539]
-
Vallerand, I. A.; Patten, S. B.;Barnabe, C. Curr. Opin. Rheumatol. 2019, 31, 279–284.
[https://doi.org/10.1097/BOR.0000000000000597]
-
Koo, B.; Bae, H. J.; Goo, N.; Kim, J.; Kim, J.; Cai, M.; Jung, I. H.; Cho, K.; Jung, S. Y.; Chang, S. W.; Jang, D. S.; Ryu, J. H. J. Pharm. Pharmacol. 2020, 72, 149–160.
[https://doi.org/10.1111/jphp.13199]
-
Jeong, Y.; Bae, H. J.; Park, K.; Bae, H. J.; Yang, X.; Cho, Y.-J.; Jung, S. Y.; Jang, D. S.; Ryu, J. H. J. Ethnopharmacol. 2022, 285, 114864.
[https://doi.org/10.1016/j.jep.2021.114864]
-
Jung, J. M.; Park, S. J.; Lee, Y. W.; Lee, H. E.; Hong, S. I.; Lew, J. H.; Hong, E.; Shim, J. S.; Cheong, J. H.; Ryu, J. H. J. Ethnopharmacol. 2013, 148, 826–834.
[https://doi.org/10.1016/j.jep.2013.05.019]
-
Butelman, E. R.; McElroy, B. D.; Prisinzano, T. E.; Kreek, M. J. J. Pharmacol. Exp. Ther. 2019, 370, 1–8.
[https://doi.org/10.1124/jpet.119.256354]
-
Khemissi, W.; Farooq, R. K.; Le Guisquet, A.-M.; Sakly, M.; Belzung, C. Fron.t Behav. Neurosci. 2014, 8, 340.
[https://doi.org/10.3389/fnbeh.2014.00340]
-
Yi, S.-S.; Zou, J.-J.; Meng, L.; Chen, H.-M.; Hong, Z.-Q.; Liu, X.-F.; Farooq, U.; Chen, M.-X.; Lin, Z.-R.; Zhou, W.; Ao, L.-J.; Hu, X.-Q.;Niu, L.-L. Front. Psychiatry. 2022, 13, 864481.
[https://doi.org/10.3389/fpsyt.2022.864481]
- de Araújo, A. B.; Azul, F. V. C.; Silva, F. R. M.; de Almeida, T. S.; Oliveira, J. V. N.; Pimenta, A. T. T.; Bezerra, A. M. E.; Machado, N. J.; Leal, L. K. A Oxid. Med. Cell Longev. 2022, 2022, 6304087.
-
Benros, M. E.; Waltoft, B. L.; Nordentoft, M.; Ostergaard, S. D.; Eaton, W. W.; Krogh, J.; Mortensen, P. B. JAMA Psychiatry. 2013, 70, 812–820.
[https://doi.org/10.1001/jamapsychiatry.2013.1111]
-
Goldsmith, D. R.; Rapaport, M. H.; Miller, B. J. Mol. Psychiatry 2016, 21, 1696–1709.
[https://doi.org/10.1038/mp.2016.3]
-
Haapakoski, R.; Mathieu, J.; Ebmeier, K. P.; Alenius, H.; Kivimäki, M. Brain Behav. Immun. 2015, 49, 206–215.
[https://doi.org/10.1016/j.bbi.2015.06.001]
-
Howren, M. B.; Lamkin, D. M.; Suls, J. Psychosom. Med. 2009, 71, 171–186.
[https://doi.org/10.1097/PSY.0b013e3181907c1b]
-
Köhler, C. A.; Freitas, T. H.; Maes, M.; de Andrade, N. Q.; Liu, C. S.; Fernandes, B. S.; Stubbs, B.; Solmi, M.; Veronese, N.; Herrmann, N.; Raison, C. L.; Miller, B. J.; Lanctôt, K. L.; Carvalho, A. F. Acta. Psychiatr. Scand. 2017, 135, 373–387.
[https://doi.org/10.1111/acps.12698]
-
Locateli, G.; de Oliveira Alves, B.; Miorando, D.; Ernetti, J.; Alievi, K.; Zilli, G. A. L.; Serpa, P. Z.; Vecchia, C. A. D.; Mota da Silva, L.; Müller, L. G.; Roman Junior, W. A. Behav. Brain Res. 2020, 395, 112863.
[https://doi.org/10.1016/j.bbr.2020.112863]
-
Zhang, B.; Wang, P.-P.; Hu, K.-L.; Li, L.-N.; Yu, X.; Lu, Y.; Chang, H.-S. Molecules 2019, 24.
[https://doi.org/10.3390/molecules24112035]
-
Miller, B. J.; Buckley, P.; Seabolt, W.; Mellor, A.; Kirkpatrick, B. Biol. Psychiatry 2011, 70, 663–671.
[https://doi.org/10.1016/j.biopsych.2011.04.013]
-
Mongan, D.; Ramesar, M.; Föcking, M.; Cannon, M.; Cotter, D. Early Interv. Psychiatry 2020, 14, 385–397.
[https://doi.org/10.1111/eip.12859]
-
Lung, Y.-B.; Seong, S.-C.; Lee, M.-C.; Shin, Y.-U.; Kim, D.-H.; Kim, J.-M.; Jung, Y.-K.; Ahn, J.-H.; Seo, J.-G.; Park, Y.-S.; Lee, C.-S.; Roh, K.-J.; Han, C.-K.; Cho, Y.-B.; Chang, D.-Y.; Kwak, W.-J.; Jung, K.-O.; Park, B.-J. Am. J. Chin. Med. 2004, 32, 291–301.
[https://doi.org/10.1142/S0192415X04001941]
-
Nadeem, M.; Imran, M.; Aslam Gondal, T.; Imran, A.; Shahbaz, M.; Muhammad Amir, R.; Wasim Sajid, M.; Batool Qaisrani, T.; Atif, M.; Hussain, G.; Salehi, B.; Adrian Ostrander, E.; Martorell, M.; Sharifi-Rad, J.; C. Cho, W.;Martins, N. Applied Sciences 2019, 9, 3139.
[https://doi.org/10.3390/app9153139]
-
Noguchi-Shinohara, M.; Ono, K.; Hamaguchi, T.; Iwasa, K.; Nagai, T.; Kobayashi, S.; Nakamura, H.; Yamada, M. PLoS One. 2015, 10, e0126422.
[https://doi.org/10.1371/journal.pone.0126422]
-
Lataliza, A. A. B.; de Assis, P. M.; da Rocha Laurindo, L.; Gonçalves, E. C. D.; Raposo, N. R. B.; Dutra, R. C. Phytother. Res. 2021, 35, 6974–6989.
[https://doi.org/10.1002/ptr.7318]
-
Colla, A. R. S.; Pazini, F. L.; Lieberknecht, V.; Camargo, A.; Rodrigues, A. L. S. Metab. Brain Dis. 2021, 36, 437–446.
[https://doi.org/10.1007/s11011-020-00658-4]
-
Jung, Y. B.; Roh, K. J.; Jung, J. A.; Jung, K.; Yoo, H.; Cho, Y. B.; Kwak, W. J.; Kim, D. K.; Kim, K. H.; Han, C. K. Am. J. Chin. Med. 2001, 29, 485–491.
[https://doi.org/10.1142/S0192415X01000502]
-
Mikami, T.; Kim, J.; Park, J.; Lee, H.; Yaicharoen, P.; Suidasari, S.; Yokozawa, M.; Yamauchi, K. Sci. Rep. 2021, 11, 12495.
[https://doi.org/10.1038/s41598-021-90589-6]
-
Baldi, E.; Bucherelli, C. Nonlinearity Biol. Toxicol. Med. 2005, 3, 9–21.
[https://doi.org/10.2201/nonlin.003.01.002]
-
Wei, Y.; Hu, Y.; Qi, K.; Li, Y.; Chen, J.;Wang, R. Behav. Brain Res. 2022, 423, 113775.
[https://doi.org/10.1016/j.bbr.2022.113775]
-
Vratarić, M.; Šenk, V.; Bursać, B.; Gligorovska, L.; Ignjatović, D.; Kovačević, S.; Veličković, N.; Djordjevic, A. Biofactors 2021, 49, 90–107.
[https://doi.org/10.1002/biof.1802]
-
Tiwari, S.; Qi, L.; Wong, J.; Han, Z. Brain Behav. 2022, 12, e32581.
[https://doi.org/10.1002/brb3.2581]
-
Song, G.; Ouyang, G.; Bao, S. J. Cell Mol. Med. 2005, 9, 59–71.
[https://doi.org/10.1111/j.1582-4934.2005.tb00337.x]
-
Costemale-Lacoste, J. F.; Guilloux, J. P.; Gaillard, R. Encephale 2016, 42, 156–164.
[https://doi.org/10.1016/j.encep.2016.02.003]
-
Jin, T.; Zhang, Y.; Botchway, B. O. A.; Zhang, J.; Fan, R.; Zhang, Y.; Liu, X. Food Chem. Toxicol. 2022, 164, 113091.
[https://doi.org/10.1016/j.fct.2022.113091]
-
Li, M.-M.; Wang, X.; Chen, X.-D.; Yang, H.-L.; Xu, H.-S.; Zhou, P.; Gao, R.; Zhang, N.; Wang, J.; Jiang, L.; Liu, N. Behav. Brain Res. 2022, 432, 113987.
[https://doi.org/10.1016/j.bbr.2022.113987]
-
Monick, M. M.; Carter, A. B.; Robeff, P. K.; Flaherty, D. M.; Peterson, M. W.; Hunninghake, G. W. J. Immunol. 2001, 166, 4713–4720.
[https://doi.org/10.4049/jimmunol.166.7.4713]
-
Ben Menachem-Zidon, O.; Ben Menahem, Y.; Ben Hur, T.; Yirmiya, R. Neuropsychopharmacology 2015, 40, 524.
[https://doi.org/10.1038/npp.2014.264]
-
Airas, L.; Yong, V. W. Curr. Opin. Neurol. 2022, 35, 299–306.
[https://doi.org/10.1097/WCO.0000000000001045]
-
Heman-Bozadas, P.; Romero, C.; Martínez-Remedios, P.; Freitag, I.; Frías, A.; Saavedra-López, E.; Casanova, P. V.; Roig-Martínez, M.; Cribaro, G. P.; Rovirosa-Hernández, M. J.; Hernandez-Baltazar, D.; Barcia, C. J. Neuroimmunol. 2022, 367, 577874.
[https://doi.org/10.1016/j.jneuroim.2022.577874]
-
Mattoli, S.; Colotta, F.; Fincato, G.; Mezzetti, M.; Mantovani, A.; Patalano, F.; Fasoli, A. J. Cell Physiol. 1991, 149, 260–268.
[https://doi.org/10.1002/jcp.1041490212]